Diffraction of Light
In the previous lecture about light interference we considered two slits that allow a flat wave front to go through and considered the light coming out from these two slits as two sources of light in phase with each other.
We assumed that these two slits were of infinitely small width, so we can only consider interaction between them, not paying attention to interaction of light rays coming into and from one single slit.
Since any slit has a finite width, according to the Huygens Principal, all points of a wave front reaching a slit are independent sources of light. Rays, coming in phase to a slit, go through, producing waves in all directions on exit from a slit, and these secondary waves of light will interfere with each other.
Generally, any wave front, according to the Huygens Principle, is a set of independent points that are sources of secondary light rays that, potentially, interfere with each other.
This process of interference between the neighboring rays of light is called diffraction.
Imagine a set of parallel rays of light going through a perpendicular to their direction slit and, after passing through it, falling onto a perpendicular to their direction screen positioned at some distance from a slit.
The first what we notice on a screen is a bright line that corresponds to a slit the light goes through.
But, if we look more attentively, we would notice the parallel lines on both sides of the bright line, which we can only attribute to the interference among light rays coming out of a slit.
The picture that might be observed would look similar to this:
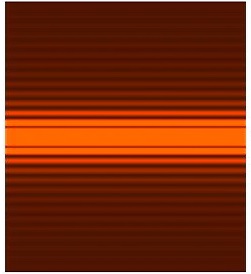
The lines parallel to the central bright line are the result of interference among light rays going through one and only slit of a small but finite width. This is called the diffraction of light.
Our task is to understand how the diffraction works, applying appropriate approximations to shorten the calculations, as physicists usually do.
For this purpose we choose a single point S on a screen at a distance x from a center of a main bright line and examine all light rays that go from a slit to this point, as presented on a picture below.
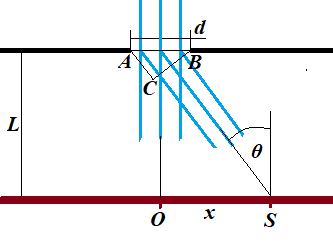
Let the screen's distance from a slit be relatively large L (say, about 1 meter) and the width of a slit be relatively small d, like 1 micrometer or so, which is comparable to a visible light wave length.
Assume that monochromatic laser ray (for example, blue) goes through this slit.
Every point of observation on a screen S receives a set of light rays from all points of a wave front AB across a slit's width, that we can approximately consider as set of parallel rays because the distance L is significantly larger than d.
To determine the amount of light falling onto any particular point of observation, like O or S, we have to consider only these approximately parallel light rays coming from the slit to the point of observation and interactions between them, disregarding all other rays emitted to other directions from the slit.
The incident angle of the rays falling perpendicularly to a screen from a slit to point O equals, approximately, to zero. All the rays falling into this point from a slit are covering, approximately, the same distance L to reach this point.
Being in phase at a slit, all these light rays will be in phase at point O.
Therefore, all the rays falling into point O will interfere constructively with each other, they will all contribute light energy, and that's the reason for the bright image of a line to be present on the screen at this point.
The picture at other points, not on a perpendicular from a slit to a screen, is shown on the example of point S.
Different light rays falling from a slit onto point S will have to cover different distances because of finite width of a slit.
The distance AS is longer than BS by
Δ = √L²+(x+(d/2))² −
− √L²+(x−(d/2))²
Usually, this complicated formula is approximated, assuming that the width of the slit d=AB is small relative to a distance between a slit and a screen L.
Let p=√L²+(x+(d/2))²
and q=√L²+(x−(d/2))².
Then the following equalities are valid:
Δ = p − q
Multiply and divide this expression by a sum of these two radicals p+q:
Δ = (p² − q²)/(p + q)
p² − q² = 2x·d
p + q ≅ 2√L²+x²
Therefore,
Δ ≅ 2x·d/(2√L²+x²) =
= d·(x/√L²+x²)
But x/√L²+x² is sin(θ), where θ is an incident angle of a ray coming from a slit to a point of observation.
Therefore, approximate value of the difference between the longest distance AS from a slit to point S and the shortest one BS is
Δ ≅ d·sin(θ)
All rays between AS and BS have to cover different distances to point S greater than BS, but less than AS.
This causes all these rays to be more or less out of phase and interfere with each other in a complex constructive or destructive ways.
There is one and only one condition, when point S would be relatively dark. This happens if all the rays coming to point S from all points on the wave front AB are interfering destructively with each other, that is for each ray of light reaching point S there is one light ray reaching point S in anti-phase.
If there are some light rays emitted by a wave front AB, for which there are no anti-phase pairs to destructively interfere with them, the point S will have some light, more or less, depending on how many rays of light are not paired with anti-phase rays and, therefore, not canceled by destructive interference.
Assume the wave length of the monochromatic light falling on the slit is λ.
The first case we consider is the one when the longest distance AS from a slit to point S is by Δ=d·sin(θ)=λ greater than the shortest distance BS.
That means, ray AS is longer than BS by the wavelength λ.
If we consider points of the wave front from A to B, the distance from these points to the point of observation S will be greater than the shortest distance BS by some value that monotonously changes from maximum λ to minimum 0.
Somewhere in between A and B there will be a point M such that MS is greater than BS by half the wavelength λ/2.
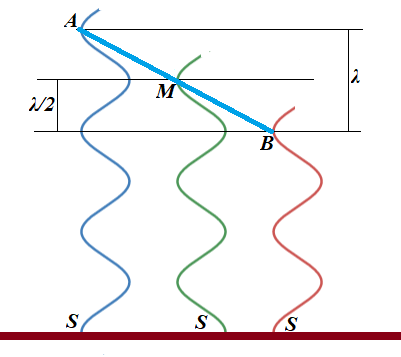
Obviously, two initially in-phase rays, BS and MS that cover distances to the same point S that differ by half a wavelength λ/2, will be in anti-phase upon arriving to that point and should cancel each other.
Our next step is to organize rays in pairs of mutually canceling rays of light and see if there are some remaining lights not canceled by a corresponding ray in anti-phase.
Let's pair a ray BS with a ray MS, where M, as mentioned above, is a point, from which the distance MS is by λ/2 greater than BS.
Since MS is longer than BS by λ/2 and these rays initially (at points M and B) were in-phase, they arrive to point S in anti-phase and cancel each other, as seen on a picture above.
Let's move slightly from point B to point B' along a wave front towards A. The distance B'S would be slightly longer than BS.
Since the distance to point S monotonously increasing as we move from B to A along the wave front from the length of BS to the length of AS, which is by λ longer, we can find a point M' slightly towards A from point M such that the distance M'S is greater than B'S by the same half wavelength λ/2.
Let's pair light rays B'S and M'S. Upon arriving to point S they will be in anti-phase and interfere destructively, that is cancel each other.
Actually, for every point B' from B (inclusive) to M (not inclusive) along the wave front we can find a corresponding point M' along a wave front from M (inclusive) to A (not inclusive) such that the difference between the distances B'S and M'S will be exactly λ/2, so the corresponding rays B'S and M'S going towards point S will arrive in anti-phase and cancel each other.
It means that all rays from every point on a wave front, except point A, will destructively interfere with each other.
The infinitesimal by amount of light ray AS, for which there is no pair in this logic, can be disregarded, as not delivering any noticeable energy to point S. There will be practically no light at point S positioned in such a way that
AS − BS = λ ≅ d·sin(θ).
The equality above allows to calculate the distance Rdark_1 of the first dark line from the bright image of the slot on the screen:
sin(θ) ≅ λ/d
Since for small θ
sin(θ) ≅ tan(θ) = Rdark_1/L,
Rdark_1 ≅ L·λ/d
Let's try to calculate the distance to the second dark line on a screen from a central bright line, using similar logic.
Imagine that
d·sin(θ) ≅ 2λ
Now, instead of breaking all the light rays into two groups, pairing each ray from the first group with the one from the second that travels the distance to point of observation S by λ/2 longer, which caused it to be in anti-phase with the first ray, effectively canceling both rays, we can divide all rays into four groups:
Group I are all those rays that go along paths longer than BS by no more than λ/2.
Group II are all those rays that go along paths longer than BS by more than λ/2, but no more than λ.
Group III are all those rays that go along paths longer than BS by more than λ, but no more than 3λ/2.
Group IV are all those rays that go along paths longer than BS by more than 3λ/2, but no more than 2λ.
All rays going through a slit would fall into one or another of these four groups.
Using the monotonic behavior of the length of a ray from a point on a wave front AB, from which it emitted, to the observation point S, we conclude that
(a) for each ray from the Group I there is one and only one ray from Group II, whose length is greater exactly by λ/2;
(b) for each ray from the Group III there is one and only one ray from Group IV, whose length is greater exactly by λ/2;
Therefore, each ray will be paired with another, whose length is longer by λ/2 and, correspondingly, which will come to point S in anti-phase, canceling both rays in a pair.
The condition
sin(θ) ≅ 2λ/d
Since for small θ
sin(θ) ≅ tan(θ) = Rdark_2/L,
Rdark_2 ≅ 2L·λ/d
It's easy to generalize now that the condition for a dark line is
d·sin(θ) ≅ N·λ
where N - some integer number. For positive N we will get the location of dark lines on one side of the middle bright image of a slot, for negative N we will get symmetrical dark line on the other side from a center.
Obviously, this condition necessitates the maximum value for N to be such that sin(θ) does not exceed 1, that is
sin(θ) = N·λ/d < 1 or
N < d/λ
In-between the dark lines there must be bright lines. They appear, when the condition
Depending on exact value of incident angle θ (or, which is equivalent, on a value of distance x from the center of picture to the observation point S), more or less rays will remain not canceled by another ray in anti-phase with it, or partially interfere destructively, retaining some light energy to light up point of observation S.
For example, consider situation:
d·sin(θ) ≅ 3λ/2
Divide all rays into three groups:
Group I are all those rays that go along paths longer than BS by no more than λ/2.
Group II are all those rays that go along paths longer than BS by more than λ/2, but no more than λ.
Group III are all those rays that go along paths longer than BS by more than λ, but no more than 3λ/2.
As before, for each ray from the Group I there is one and only one ray from Group II, whose length is greater exactly by λ/2, which will cause it to be in anti-phase with the first ray and cancel it at point S.
But for any ray in Group III there will be no pair in anti-phase. There will be rays out of phase, they will somehow interfere with each other in partially constructive or partially destructive way, but they will not completely cancel each other, some light from each ray of this group will reach point S and we will observe some light there.
Generally, the brightest lines will be observed when
d·sin(θ) ≅ (N+0.5)·λ
because for each ray of the length longer than the length of ray BS by no more than N·λ there will be a canceling ray coming to point S in anti-phase,
but for all rays of the length longer than the length of ray BS by more than N·λ there will be no canceling pairs, they will bring light to point S.
It should be noted that all the above calculations were intentionally simplified using typical approximations customary in physics.
It was sufficient for our purpose to demonstrate the qualitative picture of diffraction as the light phenomena.
More precise calculations could have been done, if more practical goals would be our aim.
No comments:
Post a Comment