Rope Waves
Waves on a rope are a more complex kind of waves than simple harmonic oscillations of a point mass on a spring.
Primary difference is that spring oscillations are longitudinal, that is each part of a spring moves along the direction of the wave propagation, while the oscillations of a rope are transverse, that is each piece of a rope moves perpendicularly to the propagation of waves on it.
To analyze these waves we will use the following model.
We assume that the rope we deal with is initially stretched along some line (we will call it "horizontal") and it has one end that is harmonically moving perpendicularly to an initial stretch of a rope. That is, its "vertical" deviation from the neutral position D(t) is a sinusoidal function of time t with certain amplitude A and angular frequency ω:
D(t) = A·sin(ω·t).
The other end of a rope we presume to be far away and fixed and there is some tension T in the rope.
We also assume that there are no other forces acting on this rope, including gravity.
Consider each infinitesimal piece of a rope as an independent point mass connected to both neighboring point masses by some links, like in a necklace.
When the edge of a rope moves up and down, each such a piece pulls its next neighbor in the same direction, as illustrated in the picture below
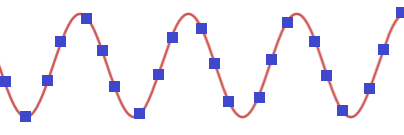
Let's introduce a system of coordinates with X-axis coinciding with the rope in its neutral position (horizontal line on a picture above) and Y-axis perpendicular to it to measure the vertical deviation of the rope's pieces from their neutral position.
If some external force moves the rope's left end from its neutral position (y=0) up to the height y=A, then down by double A (so, the end is at y=−A) and up again to return the rope's end back in the neutral position just once, the left most rope's piece will force the connected to it next one to repeat this motion by pulling the link that connects them, then next one will pull the one after etc.
A single wave (crest and trough) will go along a rope with some speed, as one rope's piece after another will repeat the up and down movement of the previous one.
To describe the dynamics of movements of each rope's piece, we will introduce a function y(x,t) - vertical displacement of a rope's piece positioned at distance x from the left end of a rope at time t passed from the beginning of this experiment.
Consider three neighboring rope's pieces with links connecting them. Assume that the left most piece pulls up the middle one and the middle one pulls up the right most.
Let the X-coordinate of the piece B be x. We are analyzing the positions of these rope's pieces at time t.
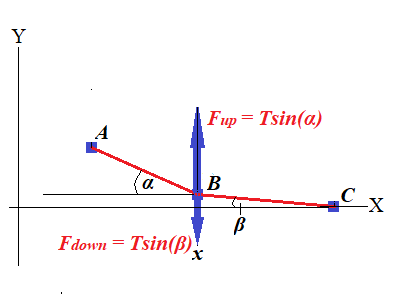
The most important force acting on all pieces is the rope's tension T, which is the same between pieces A and B as between B and C.
As piece A moves up, it pulls piece B, but to force B to move up, the piece A must be a little higher than B, so line AB should make some angle α with the horizontal level of rope's neutral position.
As piece B moves up, it pulls piece C, but to force C to move up, the piece B must be a little higher than C, so line BC should make some angle β with the horizontal level of rope's neutral position.
What's important is that angle α is greater than β because forces act in sequence, first A lifts up B and then, later, B lifts up C. So, piece A starts rising earlier than B and piece B starts earlier than C and at the same moment in time t piece A will go up more than B.
Since we are interested only in vertical component of motion of these rope's pieces, we have to consider only vertical components of the tension forces.
The vertical component of a tension T between pieces A and B pulls piece B up and equals to
Fup = T·sin(α)
The vertical component of a tension T between pieces B and C pulls piece B down and equals to
Fdown = T·sin(β)
Therefore, the resultant force pulling piece B upward is
FB = T·[sin(α)−sin(β)]
Since we found the force, it's time to use the Newton's Second Law to obtain the wave equation.
Firstly, we need a mass of a rope's piece B. Obviously, our model assumes that a rope consists of infinite number of infinitesimal pieces of the length dx each.
If the rope is uniform, the mass distribution is linear and the mass of a piece of the length dx is μ·dx, where μ is linear density of material a rope is made of (measured in kg/m in SI).
According to the Newton's Second Law, the force acting on the body equals to its mass times acceleration. Infinitesimal piece of a rope B has a length dx, its mass is μ·dx, its acceleration in the vertical direction is the second derivative of its vertical position y(x,t) by time for any fixed x.
Therefore, the force FB can be expressed as
FB = μ·dx·∂²y(x,t)/∂²t
Secondly, we have to connect expression sin(α)−sin(β) with the function y(x,t) describing the vertical location of a rope's piece at distance x from the rope's edge at a fixed moment of time t.
This is a purely technical calculus problem.
If piece B is, in mathematical terms, an infinitesimal interval with X-coordinates from x to x+dx, the value of
For infinitesimal interval the difference between sin() and tan() of this angle is an infinitesimal of a higher order, so we can replace
But tan(α) is the first derivative of y(x,t) by x, that is ∂y(x,t)/∂x.
An increment of the first derivative on an infinitesimal interval from x to x+dx is the second derivative times dx.
Therefore, as dx tends to zero,
sin(α)−sin(β) tends to
[∂²y(x,t)/∂x²]·dx
Now our original equation
FB = T·[sin(α)−sin(β)]
can be expressed as μ·dx·∂²y(x,t)/∂²t =
= T·[∂²y(x,t)/∂x²]·dx
Canceling dx on both sides, we obtain the following
μ·∂²y(x,t)/∂²t = T·∂²y(x,t)/∂x²
which is equivalent to this differential equation of the second order - the wave equation
(μ/T)·∂²y(x,t)/∂²t = ∂²y(x,t)/∂x²
Let's find a solution to this differential equation with initial condition describing the harmonic oscillations imposed on the rope's edge.
If we start from moving the rope's end up from a neutral position, the initial condition would be
y(0,t) = A·sin(ω·t)
If we start from moving the rope's end down from a neutral position, the initial condition would be
y(0,t) = −A·sin(ω·t)
With the initial condition of moving the rope's end up we can suggest the solution to an equation above:
y(x,t) = A·sin(ω·t−k·x)
For downward initial movement, a suitable solution can be
y(x,t) = A·sin(k·x−ω·t)
We will use the former in our calculations, but the latter one would be as good; in different textbooks you might see one or another.
Obviously, the suggested solution satisfies the initial condition:
y(0,t) = A·sin(ω·t−k·0) =
= A·sin(ω·t)
Let's check if this is a solution to a differential equation we have constructed.
We need to calculate
∂y(x,t)/∂t = A·ω·cos(ω·t−k·x)
∂²y(x,t)/∂²t =
= −A·ω²·sin(ω·t−k·x)
= −ω²·y(x,t)
∂y(x,t)/∂x = −A·k·cos(ω·t−k·x)
∂²y(x,t)/∂²x =
= −A·k²·sin(ω·t−k·x) =
= −k²·y(x,t)
From the above follows that for y(x,t)=A·sin(ω·t−k·x) it is true that
(k²/ω²)·∂²y(x,t)/∂²t = ∂²y(x,t)/∂x²
Therefore, thus chosen function y(x,t)=A·sin(ω·t−k·x) will be a solution to our differential equation, if we choose angular frequency ω and factor k such that
k²/ω² = μ/T
We can prove that the physical meaning of the ratio ω/k is the speed of a wave v, that is the speed of a crest of a wave moving along the X-axis as time t goes.
The crest of a wave A·sin(ω·t−k·x) is when function sin() reaches its maximum value of 1, which happens when its argument
Assume that for some moment in time t this crest is at point x, that is
ω·t−k·x = 2πN
Let a small amount of time Δt pass.
To find where the crest is now, that is what is an increment of the distance Δx a moving crest covered during the time Δt, we should solve the following equation for Δx:
ω·(t+Δt)−k·(x+Δx) = 2πN =
= ω·t−k·x
Opening all parenthesis, we obtain:
ω·t + ω·Δt − k·x − k·Δx =
= ω·t − k·x
Canceling identical members from left and right side, we obtain
ω·Δt − k·Δx = 0
From this follows
ω·Δt = k·Δx
Δx/Δt = ω/k
Obviously, when Δt→0,
lim Δx/Δt = v - a speed of a moving crest v.
Therefore,
v = ω/k
Consequently,
k = ω/v = (2π/T)/(λ/T) = 2π/λ
Above we have concluded that the requirement for
y(x,t) = A·sin(ω·t−k·x)
to be a solution to our differential equation is
k²/ω² = μ/T
Using the link between the speed of propagation of a wave v and the ratio of its angular frequency ω to a coefficient at the distance variable k, we can rewrite our original wave equation for a rope
(μ/T)·∂²y(x,t)/∂²t = ∂²y(x,t)/∂x²
as
(1/v²)·∂²y(x,t)/∂²t = ∂²y(x,t)/∂x²
Physical characteristics of a rope, tension T and linear mass μ, determine the speed of wave propagation v:
v² = T/μ
The angular frequency of oscillation ω can be expressed in terms of physical characteristics as
ω = k·v = k·√T/μ
The more we tighten the string on a violin (that is, increasing tension T) - the higher the frequency of oscillation and the higher sound it makes.
The heavier the string (that is, increasing linear mass density μ) - the lower sound it makes.
The wave equation has many solutions of the form
y(x,t) = A·sin(ω·t−k·x)
The exact solution in any situation is defined by initial conditions.
Also, a bit more understandable from the physical standpoint way is to express this solution in a form
y(x,t) = A·sin[k·(v·t−x)] =
= A·sin[(2π/λ)·(v·t−x)]
No comments:
Post a Comment